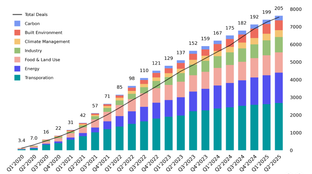
🌎 H1 2025 Climate Tech Investment: Capital stacking up for energy security & resilience
Get Sightline’s signature H1’25 investment trends report inside
Scaling long-duration energy storage lithium-ion batteries will be essential to balancing a cleaner grid
The sun doesn’t always shine and the wind doesn’t always blow, and there’s certainly no way to ensure that the brightest or breeziest times coincide with periods of peak energy demand.
The more intermittent green electrons we produce, the more we need to control when and where those green electrons are distributed in order to keep our homes and businesses humming 24-7.
Generally, once grids reach a tipping point of ~60% penetration of wind and solar energy, the capability to shift those green electrons becomes critical. Essentially we can either 1) move electrons further distances or 2) create ways to store them longer.
While transmission line upgrades provide cost savings in the long term, initial costs are high and new build of transmission is prohibited by the familiar NIMBYism and permitting challenges. With limited transmission infrastructure, smoothing out the intermittency of renewables requires 12+ hour storage. Technologies able to store energy from ~8hrs up to multiple days or weeks are categorized as long duration energy storage (LDES).
Along with enabling a cleaner grid, LDES tech provides greater energy resilience and reliability. The increasing frequency and length of PG&E’s public safety power shutoffs during extreme heat and weather events in California, for example, creates a recurring need for multi-day energy storage—especially in the commercial and industrial sector, where not all customers are considered “essential” but may still have needs like manufacturing or cold storage that can no longer be met economically or sustainably with diesel generator backups.
The grid and energy markets have a fundamental responsibility: perfectly matching energy generation with demand in a 100% safe manner for all customers, every day, forever. The way that this system is regulated and managed is multi-layered (regulatory commissions, system operators, utilities, and more!) and complex enough for its own deep dive, but at the core are two ways of provisioning and compensating the folks who produce electrons in deregulated systems: capacity markets and energy markets.
Capacity markets operate based on long-term models of how much power the grid will need at any given instant under varying conditions in order to meet demand, and contracts are paid ahead of time to energy generators for their ability to deliver that power when needed—typically years in advance.
Energy markets are a real-time mechanism of compensating generators for delivering energy to the grid in sync with demand on a daily, hourly, and momentary basis. Since load-serving entities and grid operators have a pretty good idea of how much energy will be needed 24 hours ahead of time, the bulk of transactions pay for the next day’s energy production. More instantaneous energy transactions compensate for small variabilities in grid conditions, as well as “ancillary services,” like making sure that voltage and frequency stay stable at all times.
Storage assets even out imbalances and generate revenue by charging up with electrons when there’s an abundance of renewable energy, then selling it back to the grid when demand and prices are higher. They can also claim capacity market revenues and be compensated for providing ancillary services such as frequency regulation to the grid as well.
Depending on their duration, LDES technologies are best-suited for different problems. Lithium-ion batteries are typically most economical for between one and eight hours, while a collection of novel solutions are targeting the 12-24 hour range. Other technologies can store energy with minimal losses over weeks or even months, providing balance to the grid during storms or between seasons.
LDES solutions have a long (and somewhat tumultuous) history:
Flow battery form factors and vanadium chemistries are well known and had a brief boost in popularity in the 2000s and 2010s during cleantech 1.0. Excitement about the technology resurfaced ~5 years ago and grew with the IPO of ESS, but the technology hasn’t quite reached its flow state for cost reductions—43% of flow battery companies founded between 2000 and 2010 are no longer in business.
While flow batteries promise the holy grail of decoupling of storage and power in battery systems—a major drawback of scaling li-ion batteries (see below), the technology hasn’t benefited from the growth of consumer EV production that has pushed li-ion chemistries down the cost curve.
Most energy storage solutions today rely on lower-cost li-ion batteries (typically LFP), which have high energy density, making them small enough to be placed just about anywhere. Scaling is a relatively simple process of adding more containerized units, and as li-ion supply chains are gearing up full-force for EVs, li-ion battery costs have plummeted. But li-ion batteries can’t solve all our energy storage problems.
While li-ion batteries are great for short-term balancing and peak shifting, they’re not so good at storage across days, weeks, or seasons.
Energy storage is driven by two key concepts: energy capacity and charge/ discharge power capacity. In climate, (almost) everything can be simplified into a good ol’ bathtub analogy:
Traditional cell-based systems like lithium-ion batteries combine the components that store energy and produce power, and can only scale both at a fixed 1:1 ratio. In comparison, each attribute of an LDES system can be independently sized to prioritize energy storage capacity at a fixed power output. The result? A cheaper and simpler storage solution without unnecessary extra power production.
Despite the trail of failed battery ventures, funding for LDES is ramping back up, with companies like Form Energy leading the charge.
Investor interest in novel LDES technologies has returned in force driven by $100M+ mega-rounds (e.g. Form Energy, EnergyVault). Total funding increased 36x from $0.1 to $2.3B in the last five years, and 2021 in particular put a stake in the ground for the return of LDES, with funding up ~300% compared to the prior year.
While deal activity has consistently grown, it’s still early in the game. With the exception of a few break-out megarounds, ~70% of deal activity were made up of grants, Seed or Series A rounds.
As investor interest picked up, so has company development. The concentration of new company formations track with the battery market’s two active cycles: 30% of companies founded during the 2009-2011 period and 45% founded post-2017 in today’s boom. Meanwhile, the quiet period during the early 2010s was a symptom of cleantech 1.0’s fallout, which left a wake of failed battery startups.
LDES technologies generally fall into one of three categories: mechanical, electrochemical, or thermal. (There are also chemical LDES solutions, which mostly consist of hydrogen storage and aren't included in this analysis.)
Energy stored as potential or kinetic energy
This generally involves either compressing gas in order to capture the energy released as it expands, or raising up a weight and capturing energy released as it is lowered. The technologies behind this pathway are generally well understood through prior applications—air compression and lifting weights aren’t new ideas—but many companies are working on new ways of using these technologies more efficiently.
Compressed Air: Energy is used to compress air into a large underground cavern (recharge). The air is later released into a recuperator and heated for re-expansion at a turbine for generation (discharge).
Liquid Air: Sometimes called cryo-storage, air is cooled to cryogenic temperatures (recharge) with compression and cooling, then allowed to expand (discharge), using hot and cold storage tanks.
Liquid CO2: Energy is used to compress CO2 to liquid form (recharge), and then the liquid is evaporated and heated in order to expand and spin a turbine (discharge).
Gravity-based: A piston or weight is lifted using energy (recharge). When dropped, the object’s gravitational energy is captured and used to spin a turbine (discharge).
Pumped Hydropower: Configuration of two water reservoirs at different elevations, that can generate power as water moves down from one to another (discharge), passing through a turbine. The systems then uses power to pump water back into the upper reservoir (recharge). There are also new takes on this concept, where water is pumped into the subsurface and stored at high pressure until a valve on the surface is released and the fluid comes out and spins a turboexpander to produce power—examples here are Quidnet, or even Fervo with it’s recent announcement.
Batteries of different chemistries used to store energy
Electrochemical cells consist of a cathode, an anode, and an electrolyte through which a charge can pass. Significant research has gone into finding alternatives to lithium-ion batteries in this space, with varying levels of success and progress.
Metal-Air: In these systems, the anode is made of pure metal (e.g. iron) and a reaction occurs with ambient air when the metal anode is oxidized (discharge). An electrical current is then used to convert the rust back to iron (recharge).
Novel Chemistries: Battery types with a traditional anode-cathode cell structure, but utilizing alternative materials in place of lithium.
Flow batteries: A flow battery is an electrochemical cell in which two chemical components are dissolved in liquids and pumped through the system on either side of a membrane.
Using thermal energy to store and release heat and electricity
Heat can be stored in a variety of materials, including water, molten salt, and minerals. Electricity is converted to heat and used to heat up the thermal medium (recharge). That heat is then either used directly or used to boil water for a turbine (discharge). Generally converting electricity into heat and back again faces significant losses, but when the input or output of the system is heat then these technologies are far more efficient.
Latent Heat: Energy is used for phase transition of a storage medium and then reverting it to release heat.
Sensible Heat: Energy is used to heat up a storage medium (recharge). That heat is then used directly, drawn through an exchanger, or used for the expansion of another working gas/fluid (discharge).
With funding picking back up, investors can learn from the outcomes of past battery deals. These key factors will determine the success of LDES technologies.
Huge thank you to Marc Bouchet from TDK Ventures, Oliver Booth, and Francesca Gencarella for their help with research, writing, and graphics!
Get Sightline’s signature H1’25 investment trends report inside
Survey results: what’s working, what’s stalled, and what’s missing
A sneak preview from Sightline’s exclusive client-only webinar